Authors: Huber Said Padilla-Zambrano2, Douglas Carrasquilla-Franco2, Winston Eduardo Cárdenas-Chavez2, Amit Agrawal3, Tariq Janjua1, Mrinmoy Kundu4, William Andres Florez-Perdomo2, Alejandra Mendoza-Ortiz2 , Claudia Marcela Restrepo-Lugo2, Luis Rafael Moscote-Salazar2
- Regions Hospital, Saint Paul, MN, USA
- Colombian Clinical Research Group in Neurocritical Care, Colombia
- Department of Neurosurgery, All India Institute of Medical Sciences, Saket Nagar, Bhopal, Madhya Pradesh (India)
- Institute of Medical Sciences and SUM Hospital, Bhubaneswar, India
Keywords: Traumatic brain injury, Multimodal neuromonitoring, Neurocritical care, Neurotrauma, Brain function assessment
Introduction | Neuromonitoring in TBI
TBI is an acquired affection to the brain caused by an external mechanical force that can result in temporary or permanent impairment. The objective of patient management is identifying, preventing, and treating secondary brain injuries that can aggravate the patient’s outcome. The pathophysiology of brain injury requires critical evaluation and treatment, a process dependent upon multiple factors. The center point is multimodal clinical neuromonitoring, which provides oversight over the alterations in brain parameters (e.g., temperature, pressure, oxygenation) [1]. Different tools are used for multimodal neuromonitoring in TBI patients (Figure 1).
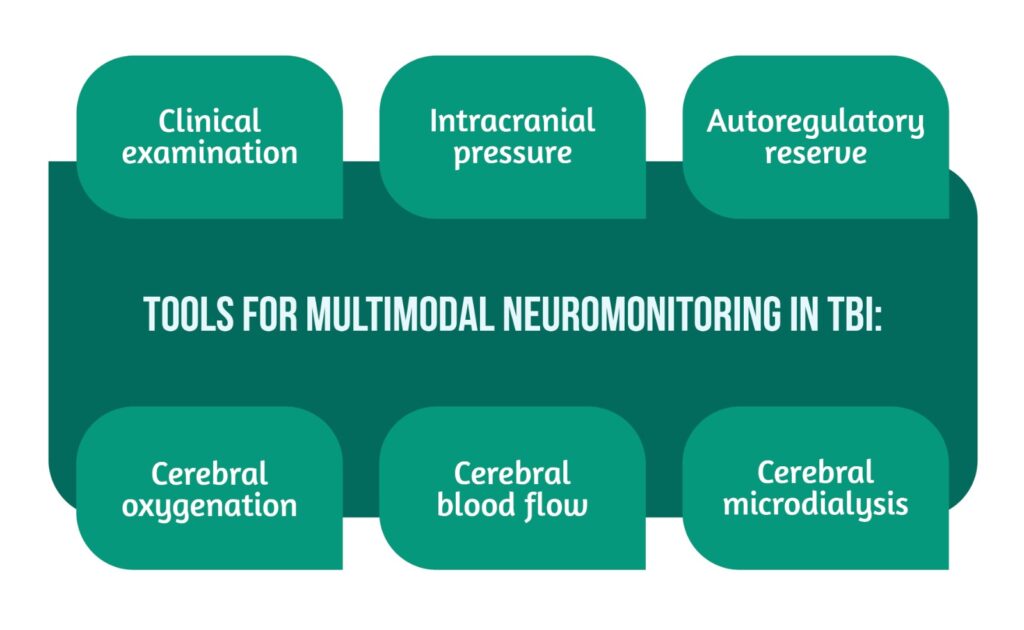
Neurological assessment
Of all the neuromonitoring modalities, the clinical examination of an awake and cooperative patient offers the most comprehensive assessment of the central nervous system (CNS) function. Despite the advances in neuroimaging and other diagnostic tools, the clinical examination performed by trained professionals is still the “Gold Standard” for assessing patients with TBI. This evaluation should include the examination of CNS structures at risk along with a comprehensive overview of several variables, including the level of consciousness (e.g., through the Glasgow Coma Scale (GCS), motor responses to verbal stimuli and pain, and the evaluation of brain stem reflexes) [2].
Despite being a fundamental component in neuromonitoring, the neurological examination has limitations that may diminish its effectiveness:
1) Patients in the Intensive Care Unit (ICU) frequently present with an altered state or with diseases that substantially limit the ability to obtain clinical information
2) Neurological evaluations are often done at different times by different examiners resulting in distinct findings and posing the issue of relevant changes going unnoticed
3) The neurological assessments are influenced by therapeutic interventions frequently done in the ICU such as endotracheal intubation, hypnotics/sedatives, analgesics, or neuromuscular blockers [2,3].
Therefore, clinical examination is insufficient to fully evaluate TBI patients and additional tests are needed.
Multimodal Monitoring
Multimodal neuromonitoring (MNM) is used complementary to clinical neurological evaluation, diagnostic imaging, and other diagnostic methods to obtain the most complete documentation of the brain physiology and pathophysiology. MNM integrates several variables, providing a better overview of the pathophysiology of the injured brain and highlighting its response to interventions.
MNM can be a valuable tool in the early detection of deterioration in neurocritical patients who are particularly vulnerable to silent cerebral infarctions. This technique involves direct tissue monitoring, achieved by inserting probes into the cerebral parenchymal tissue through a trephine orifice and fixing them in place with a cranial pin system. Monitoring brain tissue with a higher risk of secondary injury is suggested to be particularly beneficial in guiding clinicians to intervene before irreversible damage occurs [2].
The MNM integrates many devices, such as:
Technique/tool | Advantages | Limitations |
---|---|---|
Intracranial pressure monitoring | Direct measurement of pressure in the brain, a key indicator of brain health | Invasive procedure, may carry risks (e.g., infection, hemorrhage, or damage to brain tissue) |
Can guide treatment decisions and prevent secondary brain injury | Can only provide information about pressure, not other aspects of brain function | |
Cerebral blood flow monitoring | Provides information about blood flow to the brain, which is critical for brain function | Invasive procedure; may carry risks (e.g., infection, hemorrhage, or damage to brain tissue) |
Can guide treatment decisions and prevent secondary brain injury | It can be difficult to interpret, as changes in blood flow can have many different causes | |
Cerebral metabolism monitoring | Provides information about how the brain uses energy and oxygen, which can be a key indicator of brain health | Invasive procedure; may carry risks (e.g., infection, hemorrhage, or damage to brain tissue) |
It can be difficult to interpret, as changes in metabolism can have many different causes | ||
Oxygenation monitoring | Provides information about how much oxygen is being delivered to the brain, which is critical for brain function | Can be affected by many factors such as blood pressure, blood flow, and lung function |
Non-invasive procedure, which is relatively safe and easy to perform |
Table 1. Advantages and Limitations of Different Multimodal Neuromonitoring Techniques and Tools
Intracranial Pressure
Intracranial Pressure (ICP) and Cerebral perfusion pressure (CPP) are the most commonly measured physiological brain parameters in the neurocritical care and are used in many treatment algorithms.
- traumatic brain injury
- aneurysmal subarachnoid hemorrhage
- ischemic stroke
- intracerebral hemorrhage.
This extensive utilization results in a vast experience with these populations [1].
Normal ICP is 7-15mmHg in adults in the supine position, varying according to age, body position, and clinical condition [2]. The term “intracranial hypertension” depends on the pathology, but levels above 15mmHg are considered abnormal [5]. When there is an increase in brain volume, regulatory mechanisms are activated to maintain normal intracranial pressure. However, if these mechanisms are disrupted, an increase in any component of the cranial vault can result in an elevation of ICP, and compensation is necessary by reducing another component to preserve normal pressure. The cerebrospinal fluid (CSF) plays a crucial role in the compensatory space mechanism, as it can be distributed to the spinal cord reservoir [5].
The most common causes of elevated ICP are showcased in Figure 2 [1].
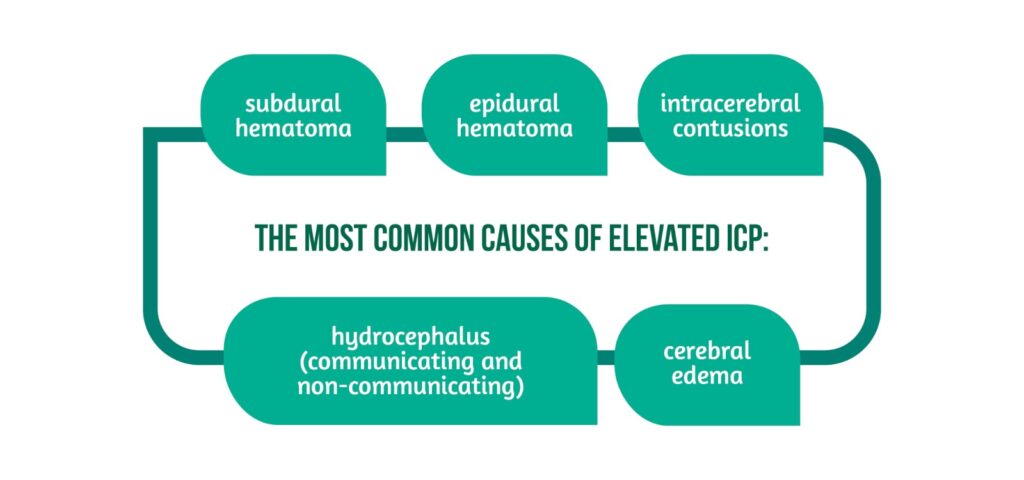
In addition, resistance to CSF flow due to brain inflammation and CSF obstruction also increases ICP, which may cause CPP reduction, and lead to secondary ischemic brain injury, brain herniation, and death if not timely managed [5].
ICP monitoring methods
ICP monitoring provides information on the state of cerebral self-regulation and is essential for the treatment of severe TBI. ICP may be measured by parenchymal microtransducers or intraventricular catheters, the latter considered the “Gold Standard” for ICP monitoring [4]. In addition, subarachnoid and epidural devices are also used. However, they are less precise [2].
Intraventricular catheters are inserted into the lateral ventricles and connected by a fluid-filled tubing system to a pressure transducer. This method allows measurement of the global ICP and allows medication administration and therapeutic drainage of CSF. Risks associated with IV catheters are hemorrhages and ventriculitis, often managed by exchanging catheters impregnated with antibiotics [8,9].
Monitoring with intraparenchymal micro transducers:
- Offers the same precision as intraventricular catheters slower infection rates
- Lower risk of hemorrhage
- Offers ease of use
- Provides continuous monitoring of the ICP.
However, this method is limited by its inability to be recalibrated [4]. Similar to clinical neuro examination, ICP monitorization alone is not sufficient to diagnose all relevant physiological brain derangements generated by brain injury.
Other noninvasive ICP monitoring techniques have been proposed as alternatives to direct ICP measurement [10] (Figure 3).
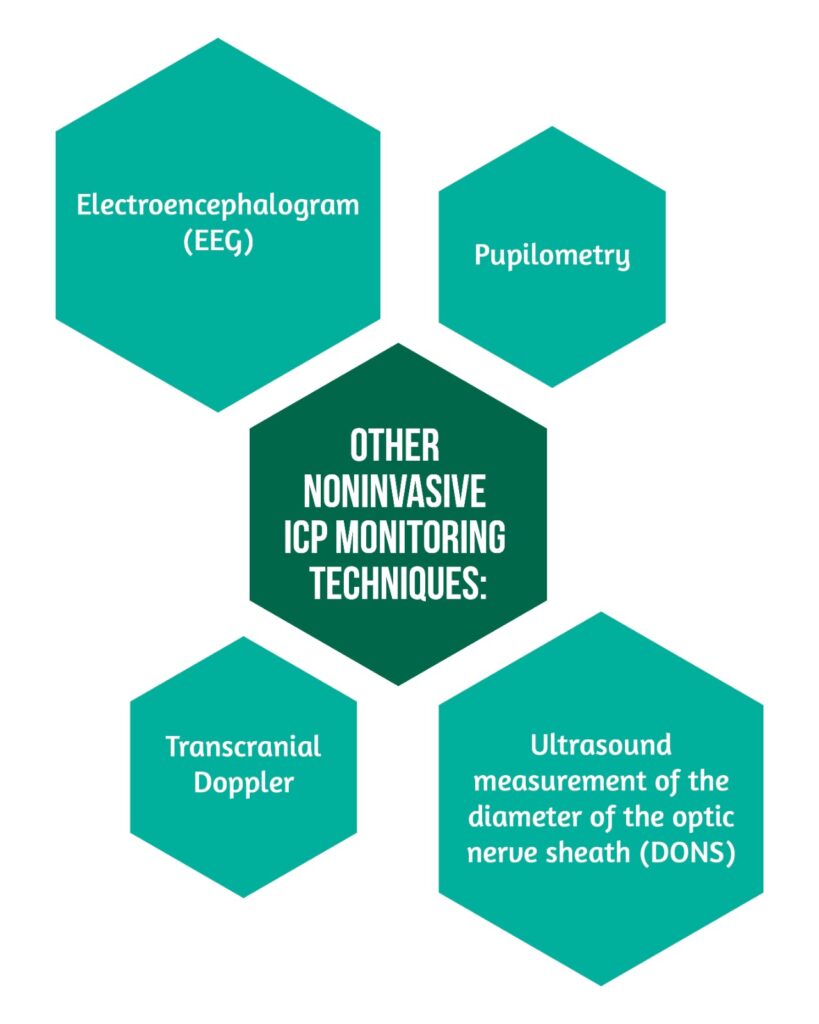
Ultrasound measurement of the diameter of the optic nerve sheath (DONS), a specialized ophthalmological method, leverages the proximity of the optic nerve sheath to the brain’s dura, which contains cerebrospinal fluid (CSF) connected to the cerebral subarachnoid space. This approach proves invaluable for indirectly identifying elevated intracranial pressure (ICP). The pupilometer measures ICP indirectly by quantitatively evaluating pupillary reflex. There is no evidence that non-invasive measures of the ICP have the same clinical utility as direct invasive monitors [10].
Cerebral perfusion pressure (CPP)
Cerebral perfusion pressure (CPP) is a surrogate measurement of cerebral blood flow, representing the difference between mean arterial blood pressure and ICP measured by invasive methods [2]. When cerebral autoregulation is normal, any increase in CPP causes compensatory vasoconstriction, reducing cerebral blood volume and ICP [1]. However, when autoregulation is lost, elevated CPP (higher than 120mmHg) can cause an increase in brain flow, leading to cerebral hyperemia, edema, and hypertensive encephalopathy [2].
Monitoring both the ICP and CPP is frequently done in patients with TBI, subarachnoid hemorrhage, and intracranial hypertension.
Vascular self-regulation
Vascular self-regulation is a fundamental brain protective mechanism from variations in cerebral blood flow, CSF, and blood pressure after TBI. This is evaluated using the index of reactivity to pressure (PRx) which is the coefficient between the mean arterial pressure (MAP) and the ICP. A negative PRx indicates a preservation of self-regulation. Alternatively, when there is an increase in the volume of the cerebral vascular compartment, the ICP is increased and a positive PRx indicates a deterioration of self-regulation [11].
Reactivity to cerebrovascular pressure is defined as the ability of vascular smooth muscle to respond to changes in transmural pressure and is determined by observing the response of ICP to changes in blood pressure. This index allows the calculation of cerebrovascular reactivity and gives value to the cerebral autoregulatory reserves [6]. In addition, cerebrovascular reactivity can be evaluated with the oxygen reactivity index which is the mobile correlation between tissue oxygen pressure (PtiO2) and PPC.
PRx is considered a global measure of self-regulatory status, while ORx represents regional self-regulation due to the focal nature of partial pressure of brain tissue oxygen (PtiO2) [9].
Cerebral blood flow (CBF)
Maintaining adequate cerebral blood flow is a fundamental therapeutic target and is quantified by neuroimaging modalities including:
- ultrasonography
- CT scan, positron emission tomography
- MRI [7].
One advantage of these techniques is their high spatial resolution. However, a limitation of these methods is that critically ill patients must be transported to the radiology department for imaging, and the information provided is limited to a single point in time, rather than continuous monitoring. The ideal neuromonitoring demands continuous measurements that can be done at the bedside [3,7].
Continuous “real-time” cerebral blood flow (CBF) monitoring is relatively new in neurointensive care and the technology is still being developed and refined. However, another modality, transcranial Doppler (TCD) has been used to evaluate focal regions of the brain with thermal diffusion flowmetry [6].
Transcranial Doppler is a non-invasive technique that measures the speed of blood flow by emitting and receiving high-frequency waves. The change in frequency that occurs as the waves pass through the blood vessels reflects the speed and direction of cerebral circulation. It can be done at the bedside but is limited to being operator-dependent [6].
Thermal diffusion fluxometry is a technique that involves using a catheter inserted into the brain’s white matter to measure the local blood flow. This catheter is equipped with two thermistors – one measures brain temperature and the other rises slightly at a temperature higher than the first [3,12]. The energy required to maintain the temperature difference between the two thermistors is proportional to the conductive properties of the heat of the white matter [12], which remain constant as long as the catheter is correctly positioned and does not move, and the convective cooling of the cerebral blood flow, which is reported in conventional units of ml/100 g/min [3].
Cerebral microdialysis: metabolic monitoring in real-time
The cerebral microdialysis is a technique that consists of extracting and quantifying the substances of the cerebral interstitial space. It is a laboratory tool introduced in the 90s and its clinical use was approved in Europe in 1995 and in the United States in 2002 [3]. The physiological premise to obtain tissue metabolites lies in the assumption that it is essential to know when the transition from an aerobic to an anaerobic metabolism occurs which results in an energy failure [1].
In practice, microdialysis focuses on markers of:
- cerebral energy metabolism (glucose, lactate, and pyruvate)
- neurotransmitters (glutamate)
- cell damage markers (glycerol).
Measurements of glucose, pyruvate, and lactate provide information on the relative contributions of aerobic and anaerobic metabolism to bioenergetics [4]. As for glycerol, it is a lipid component of neurons and its elevation is commonly associated with death or irreversible cellular ischemia. The increase in glutamate concentrations has been associated with hypoxia, ischemia, and reduced Partial pressure of oxygen in brain tissue (PbtO2) or PPC [1].
Electroencephalography
Several studies have shown that seizures are relatively common in patients with severe TBI. However, they are frequently non-convulsive, which may lead them to go unrecognized. Prophylactic anti-seizure medication is indicated for all patients with severe TBI. Seizure identification after TBI has important implications for patient m anagement and prognosis [7]. The electroencephalogram has long been used to study the electrical activity of the brain and diagnose seizures [13].
Continuous electroencephalography (cEEG) is an instrumental tool in diagnosing seizures, as delayed detection and treatment can significantly decrease the efficacy of anticonvulsant drugs [6]. Unrecognized seizures, particularly if prolonged (status epilepticus), may raise intracranial pressure, metabolic demand, excitotoxicity and worsen primary brain injury.
The limitations for the use of cEEG in the ICU include:
- The availability of trained personnel to place the electrodes
- The presence of artifacts
- The lack of trained professionals able to interpret the EEG promptly.
Additionally, there is an inherent degree of variability between the different interpretations [14]. The invasive cEEG is also available to identify crises that are not visible using scalp electrodes. Using cEEG can provide greater sensitivity to detect crises and improve the signal-to-noise ratio when compared to surface electrical activity [14].
Measuring cerebral oxygenation
Oxygenation is vital to cellular homeostasis and neuronal integrity and can be used as a marker for tissues at risk of secondary injury. PbtO2 and jugular venous oxygen saturation (SjvO2) allow continuous real-time evaluation of cerebral oxygenation. PbtO2 can:
1) provide information on oxygenation when there are normal PPC values
2) ]diagnose cerebral hypoxia secondary to low perfusion when the PPC is within normal limits.
The jugular venous oxygen saturation provides information on global cerebral oxygen. The catheter is placed in the dominant internal jugular vein and advanced to the upper part of the jugular bulb [4]. Normal values range between 55 and 75%. Lower values indicate increased oxygen extraction and risk of ischemia. The compensatory mechanism for poor oxygen supply or an increase in demand is increased oxygen extraction. In decompensated states, ischemia may occur, while high SjvO2 indicates hyperemia, decreased metabolic demand, or even cell death.
Multimodal Neuromonitoring Challenges and Limitations
MNM (multimodal neuromonitoring) has emerged as an important tool for clinicians in the management of neurocritical patients. This approach, however, is fraught with difficulties and limitations.
One significant challenge is technological limitations, as the accuracy and reliability of MNM monitoring devices can vary. This can lead to inconsistencies in data, making accurate diagnoses and treatment decisions difficult for clinicians.
Patient safety is also an important consideration, especially when invasive procedures are involved. MNM frequently necessitates the insertion of probes or sensors into the brain or surrounding tissue, which raises the risk of complications such as infection, bleeding, or damage to nearby structures [15]. To ensure patient safety, clinicians must carefully balance the potential benefits of MNM against the risks.
Cost-effectiveness and accessibility are also critical considerations. MNMs can be expensive, and their availability in some healthcare settings may be limited [16]. This can make it difficult for clinicians to gain access to these tools and provide the best possible care to patients who would benefit from MNM.
Finally, interpreting and integrating data obtained through MNM can be difficult. Clinicians must be able to interpret data from various monitoring modalities and integrate this information to form a complete picture of the patient’s condition. Specialized training and expertise are required, which may not be readily available in all healthcare settings.
Despite these obstacles, MNM remains an important tool in the care of neurocritical patients. Clinicians can provide more targeted and effective care to patients in need by carefully considering these limitations and working to overcome them.
Future Direction and Conclusions
Multimodal neuromonitoring provides valuable insights into the pathophysiology of traumatic brain injury (TBI) and helps to guide clinical decision-making and patient management. However, several areas need to be further explored, including its use in pediatric patients or those with mild TBI. Furthermore, the advancement of novel monitoring techniques, such as wearable devices, may enable continuous monitoring of brain function outside of the hospital setting. The use of machine learning algorithms to analyze large amounts of multimodal data and provide real-time decision support to clinicians is another area of future research. This has the potential to improve patient outcomes by allowing for more targeted interventions and earlier detection of secondary brain injury.
In conclusion, multimodal neuromonitoring has revolutionized the way we understand and manage traumatic brain injury. It allows early detection and compression of physiological changes caused by the injury, which will facilitate proper management of the neurocritical patient.
For more insight into neurotrauma, from the same author, visit:
- Osmotherapy in Severe TBI
- Hypothermia as a neuroprotective strategy after TBI
- Prevention of Neurotrauma is the Best Treatment
- Neurotrauma and info-pollution: A call for action
References
- Cecil S, Chen PM, Callaway SE, Rowland SM, Adler DE, & Chen JW. Traumatic brain injury: advanced multimodal neuromonitoring from theory to clinical practice. Critical care nurse 2011, 31(2), 25–37. https://doi.org/10.4037/ccn2010226
- Macas A, Bilskienė D, Gembickij A, Žarskus A, et al. Multimodal neuromonitoring. Acta Medica Lituanica 2012, 19(3), 180-186. https://doi.org/10.6001/actamedica.v19i3.2445
- Dries DJ. Textbook of Neurointensive Care. Shock. 2004;22(6):592.
- Hemphill JC, Andrews P, De Georgia M. Multimodal monitoring and neurocritical care bioinformatics. Nat Rev Neurol. 2011;7(8):451–60. DOI: 10.1038/nrneurol.2011.101
- Smith M. Monitoring intracranial pressure in traumatic brain injury. Anesth Analg. 2008;106(1):240–8. DOI: 10.1213/01.ane.0000297296.52006.8e
- Lazaridis C, Robertson CS. The Role of Multimodal Invasive Monitoring in Acute Traumatic Brain Injury. Neurosurg Clin N Am. 2016;27(4):509–17. DOI: 10.1016/j.nec.2016.05.010
- Ko S-B. Multimodality monitoring in the neurointensive care unit: a special perspective for patients with stroke. J Stroke. 2013;15(2):99–108. Available from: https://www.ncbi.nlm.nih.gov/pmc/articles/PMC3779668/
- Melhem S, Shutter L, Kaynar AM, Videtta W, et al. JOURNAL CLUB CRITIQUE A trial of intracranial pressure monitoring in traumatic brain injury. Crit Care. 2014;1–3. DOI: 10.1056/NEJMoa1207363
- Kirkman MA, Smith M. Multimodality Neuromonitoring. Anesthesiol Clin. 2016;34(3):511–23. DOI: 10.1016/j.anclin.2016.04.005
- Roh D, Park S. Brain Multimodality Monitoring: Updated Perspectives. Curr Neurol Neurosci Rep. 2016;16(6). DOI: 10.1007/s11910-016-0659-0
- Makarenko S, Griesdale DE, Gooderham P, Sekhon MS. Multimodal neuromonitoring for traumatic brain injury: A shift towards individualized therapy. J Clin Neurosci. 2016;26:8–13. DOI: 10.1016/j.jocn.2015.05.065
- Sandsmark DK, Kumar MA, Park S, Levine JM. Multimodal Monitoring in Subarachnoid Hemorrhage. Stroke. 2012;43(5):1440–5. DOI: 10.1161/STROKEAHA.111.639906
- Oddo M, Villa F, Citerio G. Brain multimodality monitoring: An update. Curr Opin Crit Care. 2012;18(2):111–8. DOI: 10.1097/MCC.0b013e32835132a5
- Korbakis G, Vespa PM. Multimodal neurologic monitoring. 1st ed. Vol. 140, Handbook of Clinical Neurology. Elsevier B.V.; 2017. 91-105 p. DOI: 10.1016/B978-0-444-63600-3.00006-4
- Kozai TD, Marzullo TC, Hooi F, et al. Reduction of neurovascular damage resulting from microelectrode insertion into the cerebral cortex using in vivo two-photon mapping. J Neural Eng. 2010;7(4):046011. DOI: 10.1088/1741-2560/7/4/046011
- Ruhatiya RS, Adukia SA, Manjunath RB, Maheshwarappa HM. Current Status and Recommendations in Multimodal Neuromonitoring. Indian J Crit Care Med. 2020;24(5):353-360. doi:10.5005/jp-journals-10071-23431