Keywords: Traumatic brain injury, TBI, Therapy, Monitoring, Critical care
Introduction | Advances in TBI care and therapies
Traumatic brain injury (TBI), a form of acquired brain injury, occurs when a sudden trauma causes damage to the brain. For example, TBI can result when the head suddenly and violently hits an object or when an object pierces the skull and enters brain tissue, representing a medical, healthcare, social, and economic burden worldwide [1].
In low-income and middle-income countries, the incidence of TBI due to traffic incidents is increasing, while in high-income countries, TBI increasingly affects older adults, primarily due to falls. However, methodological variations confound comparisons of TBI’s epidemiological patterns between regions, countries, and continents.
TBI might represent an important modifiable risk factor for
- epilepsy
- stroke
- late-life neurodegenerative disease [2].
Disabilities resulting from a TBI depend upon the severity of the injury, its location, age, and the general health of the individual.
Some common disabilities are showcased in Figure 1 [3].
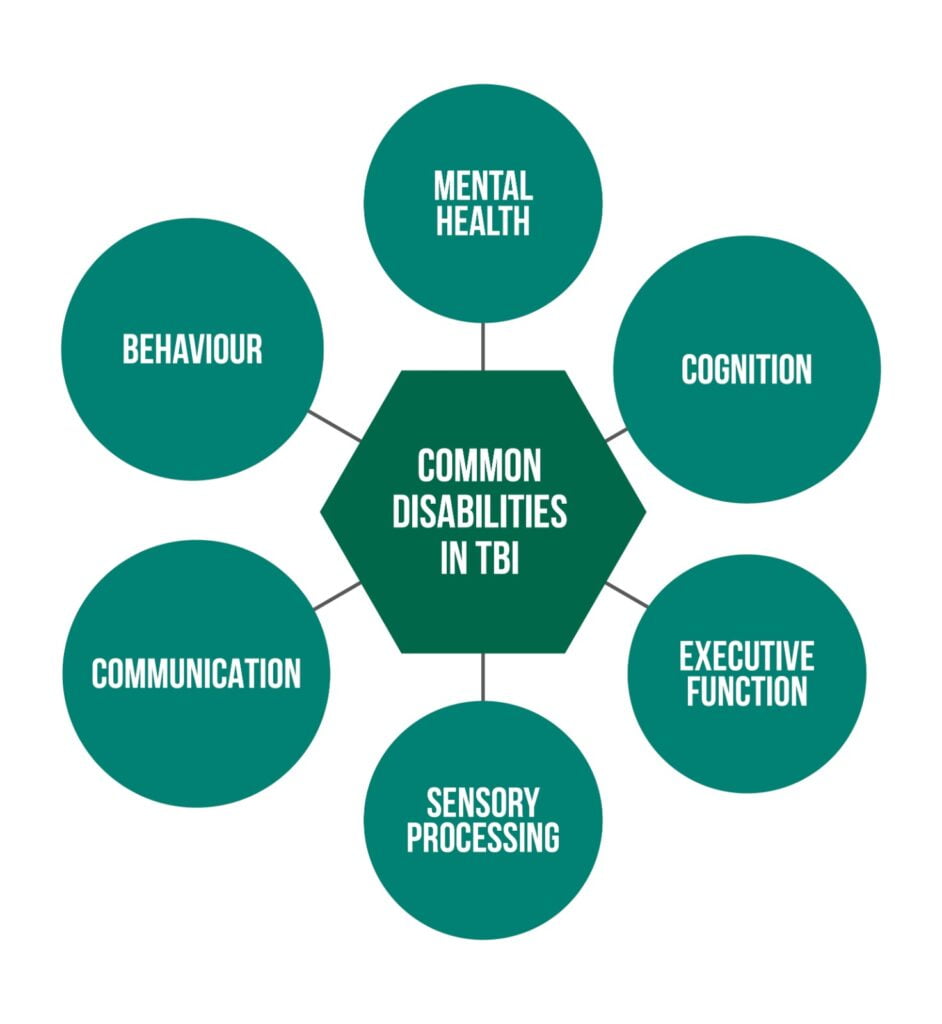
Damages of neuronal tissues associated with TBI fall into two categories: primary injury, which is directly caused by mechanical forces during the initial insult. The immediate impact of different mechanical insults on the brain can cause two types of primary injuries: focal and diffuse brain injuries. Studies have demonstrated that the co-existence of both types of injuries is common in patients who suffer from moderate to severe TBI; however, diffuse axonal injury (DAI) accounts for approximately 70% of TBI cases. Secondary injury refers to further tissue and cellular damages following the primary insult.
The biochemical, cellular, and physiological events that occur during the primary injury often progress into delayed and prolonged secondary damages, which can last from hours to years. Mechanistically, several factors contribute to secondary injuries, which include:
- excitotoxicity
- mitochondrial dysfunction
- oxidative stress
- lipid peroxidation
- neuroinflammation
- axon degeneration
- apoptotic cell death [4].
The clinical severity of TBI has long been stratified according to post-resuscitation Glasgow Coma Scale scores into mild (GCS 14–15), moderate (9–13), and severe (3–8). Severe TBI has mortality rates of 30–40% and can cause significant physical, psychosocial, and social deficits in up to 60% of cases [5].
Monitoring and neurocritical care of TBI
The cornerstone of severe TBI management is represented by monitoring and treating intracranial pressure (ICP) as an indicator of injury severity. In-hospital practical and critical care approach in patients with suspected TBI starts in the emergency department. In this phase, treatment and diagnostic assessment are done according to the Advanced Trauma Life Support (ATLS) protocol: rapid primary and secondary survey through evaluation of airways, breathing, and circulation should be performed as soon as possible during cervical spine immobilization and concomitant systemic traumatic injuries assessment [6].
The normal intracranial pressure (ICP) ranges from 7 to 15 mm Hg, while it does not exceed -15 mm Hg in the vertical position. Typically, ICP lowering therapy initiates when ICP is greater than 20 to 25 mm Hg. Refractory elevated ICP reduces cerebral perfusion pressure (CPP), thereby accounting for cerebral ischemia and initiating herniation syndromes that eventually lead to death. In addition, ICP monitoring allows for the judicious use of interventions with a defined target point, thereby avoiding potentially harmful aggressive treatment [7].
Over the past 10–15 years, ICP-based autoregulatory indices have been developed, automated ICP morphology extraction has been refined, and predictive models have been developed for clinically relevant outcomes.
However, the use of ICP is limited by several surmountable barriers:
(1) real-time or online evaluation and forecasting have not been deployed in clinical ICP monitoring and management;
(2) the information contained within frame-by-frame segmented ICP signals need to be further characterized and clinically correlated;
(3) specific morphological features need to be prioritized to maximize model fitting and allow for online clinical use;
(4) artifact detection, removal, and imputation need to be standardized to allow for the systematic study of ICP waveforms, to promote consistent online monitoring, and for robust machine learning algorithm development;
(5) model development and algorithm construction need to be refined using open-source platforms with robust versioning and regular reevaluation [8].
Increasingly sophisticated devices have been recently developed to measure oxygenation and metabolism within the brain tissue. Multimodality monitoring (MMM) is characterized by the integration and interpretation of these multiple sources of information to more comprehensively understand and monitor the brain after injury. The central use of MMM is to leverage real-time objective measurements of the brain’s mechanics, metabolism, and function to guide clinical management based on each patient’s pathophysiology and to detect physiologic alterations before secondary brain injuries occur [9].
Brain tissue oxygen (PbtO2) monitoring
Brain tissue oxygen monitoring is an emerging technology with great promise. The largest of the initial nonrandomized studies did not demonstrate a mortality benefit from PbtO2 monitoring compared with ICP monitoring but an increased hospital length of stay and poorer neurologic outcome. This may have been confounded by baseline differences in groups and implementation issues, as a subsequent meta-analysis incorporating primary data suggested a benefit. The BOOST-II (brain tissue oxygen monitoring and management in severe traumatic brain injury) trial in 2017 enrolled 117 severe TBI patients across 10 US level I trauma centers and found that a management protocol utilizing combined ICP/PbtO2 monitoring significantly reduced brain hypoxia time compared with ICP monitor-based care only.
The BOOST-II trial demonstrated feasibility, safety, and a nonsignificant trend toward decreased mortality and better 6-month neurologic outcomes. This provided the basis for the multicenter BOOST III trial, which aims to compare 6-month neurologic outcomes in approximately 1,000 patients randomized to a combined ICP/PbtO2 monitoring protocol or ICP monitor-only protocol. While its efficacy remains unproven, growing interest in this emerging technology is evident in the recent Seattle International Brain Injury Consensus Conference (SIBICC), which provides algorithmic guidelines for intervention based on combined ICP/PbtO2 monitoring, which incorporates aspects of the BOOST III protocol in a consensus fashion.
Concerning combined ICP/PbtO2 monitoring, the SIBIICC guidelines classify severe TBI patients into four categories:
- First, those with normal ICP and PbtO2 require no further treatment other than baseline neuroprotective care.
- Second, those with elevated ICP and normal PbtO2 are treated similarly to an ICP-only algorithm, but in cases of refractory ICH, more prolonged hyperventilation to 30 to 32 mm Hg may be considered.
- Third, those with normal ICP and low PbtO2 (brain hypoxia) are treated with stepwise interventions to improve this hypoxia.
- Finally, those with ICH and brain hypoxia combine these efforts, except for therapies that may harm each other, including not lowering the ICP threshold and recommending against hypercapnia.
In addition, decompressive craniotomy (DC) may be considered early intervention, as it can allow for more aggressive optimization of ICP and PbtO2 with less harmful effects on each other. These recommendations are adapted from the BOOST III protocol, and the clinical success of these management strategies remains unproven [10].
Other monitoring tools
Chemical monitoring of the injured brain can indicate tissue health and metabolism. Several markers have been used to assess inflammation, oxidative stress, excitotoxicity, and other pathophysiological mechanisms occurring within days to weeks of injury. These biomarkers have been subjected to systematic review. Notably, it has been suggested that early biomarkers of structural damage such as S-100β, GFAP, ubiquitin carboxy-terminal hydrolase L1 (UCH-L1), and tau in cerebrospinal fluid (CSF) or blood may be helpful to determine whether a head CT scan is needed after mTBI.
However, recent systematic reviews indicate there is currently only sufficient evidence to support the use of serum S100B as a biomarker of injury, while there is not enough evidence to use serum or plasma GFAP, neuron-specific enolase (NSE), UCH-L1, tau, and neurofilament proteins in this clinical application. Currently, CSF remains the most reliable biofluid for providing biomarkers of brain injury [11].
MicroRNAs (miRNAs)
MicroRNAs are short noncoding regulatory RNA molecules composed of 20-24 nucleotides that are often located within introns and play critical roles in regulating gene/protein expression. Detection of altered levels of specific miRNAs in peripheral blood and/or cerebrospinal fluid (CSF) after TBI suggests a potential use of miRNAs as injury biomarkers. They found that downregulation of miR-425-5p and miR-502 at early time points after injury characterized mild TBI patients, while upregulation of miR-21 and miR-335 was observed in serum from patients after severe TBI. Together, these studies suggest a possible biomarker application of miRNAs once their roles in TBI are better defined at the molecular and cellular levels [11].
Near-infrared spectroscopy (NIRS)
Is an emerging noninvasive monitoring modality based on chromophore absorption of infrared light with the capability of monitoring brain perfusion. NIRS could potentially address ideal neuromonitoring requirements, detect brain tissue at risk of secondary injury, and complement or even replace current invasive practices. Overall, current evidence suggests that NIRS allows intracranial bleeding detection, brain tissue oxygenation, and cerebral perfusion assessment. NIRS has also been applied to evaluate cerebral autoregulation and intracellular metabolic state during the early post-traumatic period. However, the methodological approaches between studies remain heterogeneous. As oxygenation and autoregulation seem to play a crucial role in patient treatment and outcome, the potential use of NIRS in combination with multimodal monitoring is in the best interest of both TBI patients and clinicians [12].
Seizures prophylaxis
Approximately one in five patients with severe TBI will suffer an early seizure (<7 days). High-quality evidence supports the use of antiepileptics for prophylaxis of early but not late (>7 days) seizures, initially examining phenytoin, a recommendation that exists today as per the BTF. However, many institutions have transitioned to levetiracetam, which does not require serum monitoring, is less expensive, and has more favorable side effects and drug interactions, after several studies demonstrating that it is as effective as phenytoin in preventing early seizures [10].
Therapies for TBI
The currently available clinical treatment options are described in Figure 2.
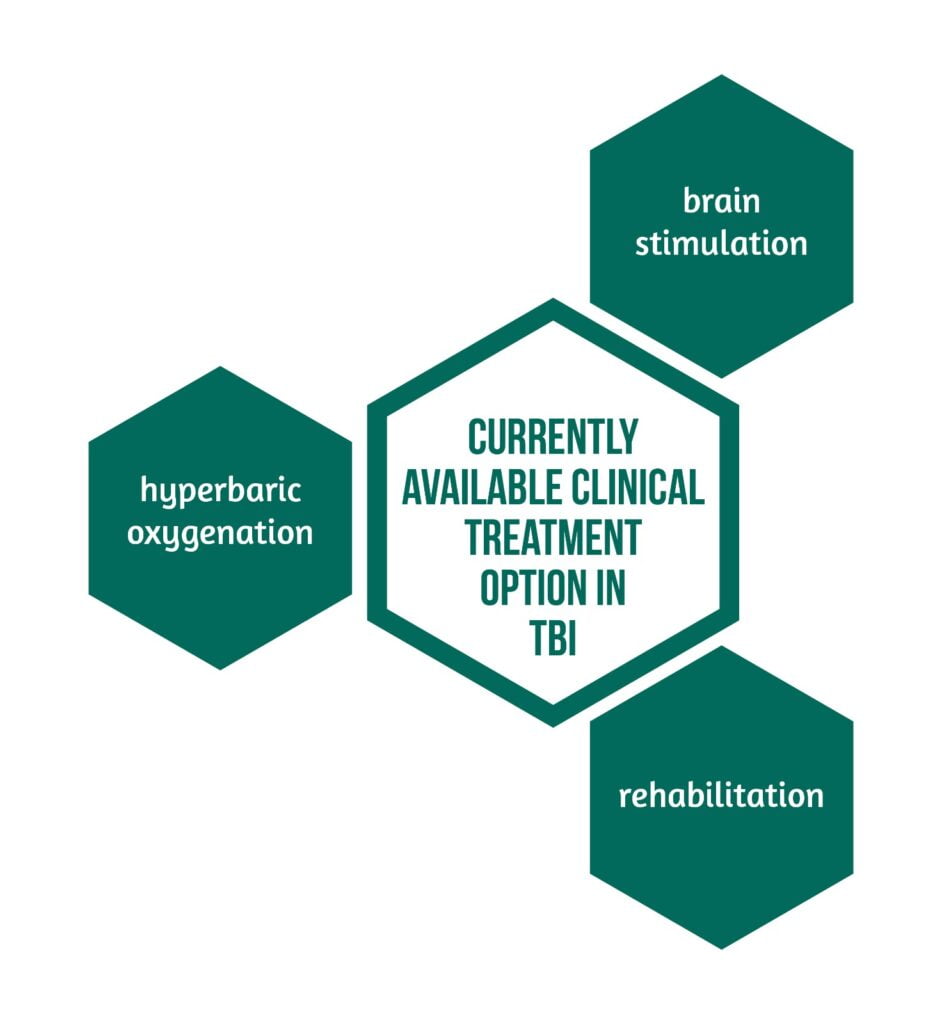
In recent years, the research on stem cell treatment of TBI has received extensive attention. Various types of stem cells, such as four types of mesenchymal stem cells, neural stem cells, and olfactory ensheathing cells, have been tried to treat TBI in clinical trials and preclinical models [4].
Hyperbaric oxygen (HBO) therapy
Is a treatment in which the patient breathes 100% oxygen while being exposed to environmental pressure >1 atmosphere absolute. HBO therapy is effective in TBI patients in terms of metabolism, oxygen toxicity, ICP, cognition, and quality of life, along with significant improvements in single-photon emission computed tomography imaging. Rockswold compared HBO and normobaric oxygen (NBO) therapy treatment effects in severe TBI and found that HBO had a more robust posttreatment effect than NBO on cerebral oxidative metabolism. In addition, in patients with mild TBI, HBO showed function alleviating the cognitive disorder after trauma, including memory, executive function, attention, and information processing speed [13].
Sensory stimulation (SS)
Is a low-invasive, safe, inexpensive, and straightforward rehabilitative approach widely studied in patients with consciousness disorders. The SS program, providing controlled exposure to sensory-specific stimulation, can facilitate recovery and prevent sensory deprivation.
The sensory stimuli are presented in Figure 3.
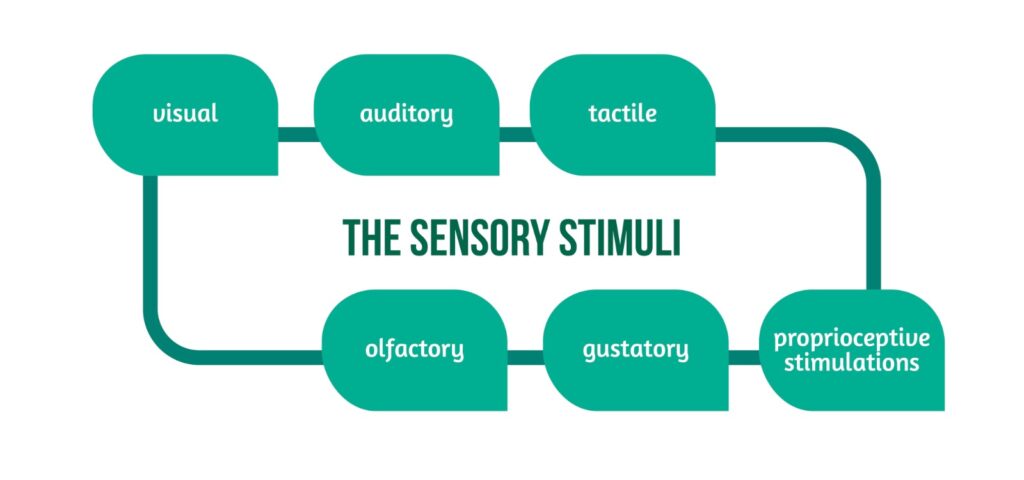
Among the coma arousal methods, SS appears to be a promising approach for recovery. Further, methodologically high-quality multicenter clinical trials or follow-up studies shall enhance evidence on the role of sensory stimulation in improving outcomes in patients with disorders of consciousness after traumatic brain injury [14].
Mesenchymal stem cells
Are multipotent, exist in adults, and can self-replicate and differentiate into various cell types. As TBI is a very complex disease and there is no effective treatment available in the clinical practice, therefore, stem cell therapy has been the focus of research in recent years. Despite great progress in animal models, the clinical translation of stem cells for TBI is limited. Mesenchymal stem cells can differentiate into neural cells, including neurons, but their differentiation without stimulation is not very effective. To improve the survival and differentiation of MSCs into nerve cells, they are usually reprogrammed before being transplanted. MSC functions are represented in Figure 4.
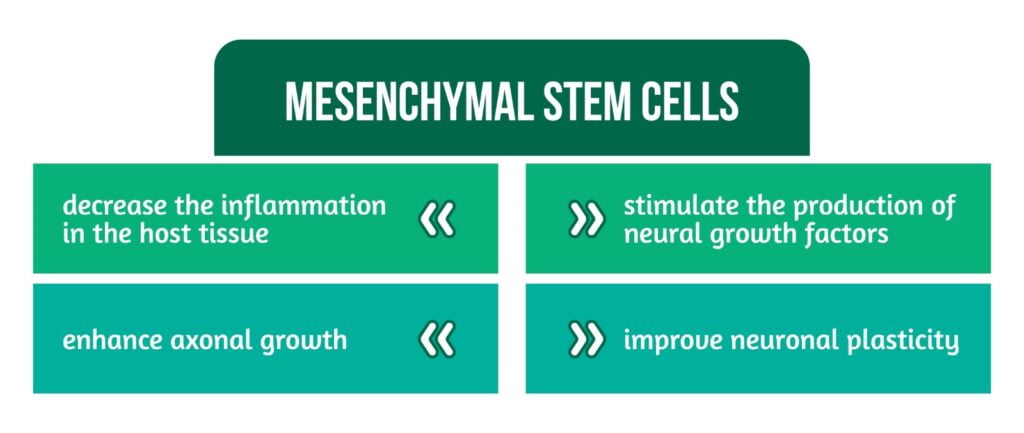
More research is still required to understand better the mode of action of MSCS and their trophic effects on traumatized host tissue [4].
Neural stem cells (NSCs)
Are self-replicating, multipotent progenitors and have the potential to differentiate into neurons, astrocytes, and oligodendrocytes. In the mouse model, it was found that the progenitor cells of NSCs were formed between 13.5 days and 15.5 days of the embryonic stage and remained inactive until birth. Two potential niches host NSCs in the adult mammalian brain, the subventricular zone (SVZ) of the lateral ventricle and the subgranular zone (SGZ) of the hippocampus. Although transplanted embryonic or adult NSCs can survive, migrate, differentiate, and improve neurologic and cognitive functions in preclinical TBI models, the realistic and ethical sources of NSCs for autologous clinical translation have not yet been achieved [4].
Olfactory ensheathing cells
(OECs) are individual glial cells that protect and support the regeneration of olfactory receptor neurons (ORNs) from the peripheral olfactory epithelium to the glomeruli of the olfactory bulb in the central nervous system. Studies have shown that co-transplantation on rats of MSCs and OECs or NSCs and OECs is more effective than transplantation of OECs alone in the treatment of rat TBI models. This might be due to the special biological features of OECs and their accompanying MSCs, which affect the regeneration of axons [4].
Conclusions
TBI is a major global health challenge and priority. At present, there is no effective treatment for TBI in clinical practice. Currently, available therapeutic options are hyperbaric oxygen, brain stimulation, and rehabilitation, as highlighted by the research of Khellfar et al. Recent developments in stem cell therapy have left a promising future for eliminating this fatal health problem. Although preclinical models have made significant progress, clinical translation is still limited.
For more information about the impact of TBI visit:
- Assessment of Quality of Life after TBI using QOLIBRI-OS
- Can TBI affect taste and smell?
- TBI outcomes in Europe
We kindly invite you to browse our Interview category https://brain-amn.org/category/interviews/. You will surely find a cluster of informative discussions with different specialists in the field of neurotrauma.
References
- The National Institute of Neurological Disorders and Stroke; Maryland; Brain Injury; 2022 July 25; Available from: https://www.ninds.nih.gov/health-information/disorders/traumatic-brain-injury
- Maas AIR, Menon DK, Adelson PD, et al. Traumatic brain injury: integrated approaches to improve prevention, clinical care, and research. Lancet Neurol. 2017;16(12):987-1048. doi:10.1016/S1474-4422(17)30371-X
- The Mayo Clinic; Minnesota; Traumatic brain injury, 2021 February 4; Available from: https://www.mayoclinic.org/diseases-conditions/traumatic-brain-injury/symptoms-causes/syc-20378557
- Alizada M, Lin S, Gao H. Recent advances in the treatment of traumatic brain injury with autologous and non-autologous multipotent stem and progenitor cells: preclinical models and clinical trials. Folia Neuropathol. 2021;59(3):298-316. doi: 10.5114/fn.2021.108536.
- Khellaf A, Khan DZ, Helmy A. Recent advances in traumatic brain injury. J Neurol. 2019;266(11):2878-2889. doi: 10.1007/s00415-019-09541-4.
- Scerrati A, De Rosa S, Mongardi L, Cavallo MA, Trapella G, De Bonis P. Standard of care, controversies, and innovations in the medical treatment of severe traumatic brain injury. J Neurosurg Sci. 2018 Oct;62(5):574-583. doi: 10.23736/S0390-5616.18.04462-4.
- Munakomi S, M Das J. Intracranial Pressure Monitoring. [Updated 2022 Jun 25]. In: StatPearls [Internet]. Treasure Island (FL): StatPearls Publishing; 2022. Available from: https://www.ncbi.nlm.nih.gov/books/NBK542298/
- Dai, Honghao; Jia, Xiaodong; Pahren, Laura; Lee, Jay; Foreman, Brandon. Intracranial Pressure Monitoring Signals After Traumatic Brain Injury: A Narrative Overview and Conceptual Data Science Framework. Frontiers in Neurology 2020, 11, 959–. doi:10.3389/fneur.2020.00959
- Foreman B, Lissak IA, Kamireddi N, et al. Challenges and Opportunities in Multimodal Monitoring and Data Analytics in Traumatic Brain Injury. Curr Neurol Neurosci Rep 21, 6 (2021). https://doi.org/10.1007/s11910-021-01098-y
- Rakhit S, Nordness MF, Lombardo SR, Cook M, Smith L, Patel MB. Management and Challenges of Severe Traumatic Brain Injury. Semin Respir Crit Care Med. 2021;42(1):127-144. doi: 10.1055/s-0040-1716493.
- Najem, Dema; Rennie, Kerry; Ribecco, Maria; Ly, Dao; Haukenfrers, Julie; Liu, Qing; Nzau, Munyao; Fraser, Douglas; Bani, Mahmud. Traumatic Brain Injury: Classification, Models and Markers. Biochemistry and Cell Biology 2018, bcb-2016-0160–. doi:10.1139/bcb-2016-0160
- Roldán M, Kyriacou PA. Near-Infrared Spectroscopy (NIRS) in Traumatic Brain Injury (TBI). Sensors (Basel). 2021;21(5):1586. doi: 10.3390/s21051586.
- Deng Z, Chen W, Jin J, Zhao J, Xu H. The neuroprotection effect of oxygen therapy: A systematic review and meta-analysis. Niger J Clin Pract. 2018;21(4):401-416. doi: 10.4103/njcp.njcp_315_16.
- Li J, Cheng Q, Liu FK, Huang Z, Feng SS. Sensory stimulation to improve arousal in comatose patients after traumatic brain injury: a systematic review of the literature. Neurol Sci. 2020;41(9):2367-2376. doi: 10.1007/s10072-020-04410-9.